Tritrophic interactions in plant defense
Tritrophic interactions, as they relate to plant defense against herbivory, describe the ecological impacts of three trophic levels on each other: the plant, the herbivore, and its natural enemies. They may also be called multitrophic interactions when further trophic levels, such as soil microbes, or hyperparasitoids (higher-order predators), are considered.[1] Tritrophic interactions join pollination and seed dispersal as vital biological functions which plants perform via cooperation with animals.[2]
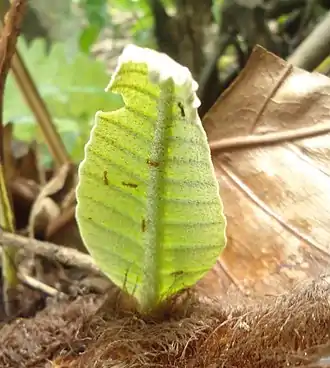
Predators, pathogens, and parasitoids that attack plant-feeding insects referred to as natural enemies in a tritrophic context; they can benefit plants by hindering the feeding behavior of the harmful insect. It is thought that many plant traits have evolved in response to this mutualism to make themselves more attractive to natural enemies. This enlisting of natural enemies functions to protect against excessive herbivory and is considered an indirect plant defense mechanism.[2] Traits attractive to natural enemies can be physical such as in the cases of domatia and nectaries,[1] chemicals, or in the case of induced plant volatile chemicals used by natural enemies to pinpoint a food source.[3] There are also numerous other plant traits that influence the success of different natural enemies in controlling herbivores which still require further investigation.[4]
This article will discuss the chemistry by which plants attract natural enemies to kill plant herbivores; it will also illustrate the alternative morphological means by which plants attract natural enemies and will explain what value in understanding these interactions can have for humans.
Chemical mechanisms of enemy attraction
Plants universally produce secondary metabolites known as allelochemicals. These metabolites serve no purpose in basic metabolic processes rather they serve as mediators of interactions between a plant and its environment,[5][6] often attracting, repelling, or poisoning insects. Not only this, they also aid in helping produce secondary cell wall components such as those produced via amino acid modification.[7] In a tritrophic system, volatiles are readily produced into the air and are superior to surface chemicals in drawing foraging natural enemies from afar. Plants also produce root volatiles which will drive tritrophic interactions between below-ground herbivores and their natural enemies.[8] A very small fraction of plant volatiles are detectable by humans which will give plants like basil, eucalyptus, and pine trees their distinctive odors.[1] The mixture and ratios of individual volatiles emitted by a plant under given circumstances is referred to as a volatile profile (also referred to as synomones in the context of natural enemy attraction). These are highly specific to certain plant species and are detectable within several meters of the source. Predators and parasitoids exploit the specificity of volatile profiles to navigate the complex infochemical signals presented by plants in their efforts to locate a particular prey species.[1]
The production of volatiles is likely to be beneficial given two conditions: that they are effective in attracting natural enemies and that the natural enemies are effective in removing or impeding herbivores. However, volatile chemicals may not have evolved initially for this purpose as they play vital roles within-plant signaling,[9] attracting pollinators,[10] or repelling herbivores that dislike such odors.[1]
Induced defenses
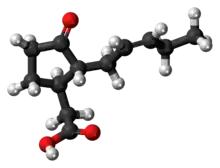
When plants continuously release a baseline level of volatiles, these defenses are said to be constitutive. Upon an instance of herbivory, however, the plant may respond by considerably increasing its production of volatiles or by producing a new profile of volatiles. This plasticity is controlled by either the jasmonic acid pathway or the salicylic acid pathway, depending largely on the herbivore; these substances are often called herbivore-induced plant volatiles (HIPVs).[1][3] Specifically, the plant hormone, jasmonic acid, increases in concentration when plants are damaged and are responsible for inducing the transcription of enzymes that are necessary in secondary metabolite production pathways.[7] In addition to its benefits in resistance and transcription, this hormone has been found to aid in the production of defensive proteins such as α-amylase inhibitors as well lectins. Since α-amylase has been known for having hydrolytic properties in its ability to break down starch, these inhibitory proteins prevent insects from properly breaking down starch.[7] Contrastingly, lectins provide their own defense benefits for plants as they interfere with insect nutrient absorption as they bind to carbohydrates.[7] Though volatiles of any kind have an attractive effect on natural enemies, this effect is stronger for damaged plants than for undamaged plants,[1] perhaps because induced volatiles signal definitive and recent herbivore activity. The phenomenon of inducibility gives rise to the idea that plants are sending out a "distress call" to the third trophic level in times of herbivore attack.
Natural enemies can distinguish between mechanical tissue damage, which might occur during a number of events other than herbivory, and damage that is the direct result of insect feeding behavior. The presence of herbivore saliva or regurgitant mediates this differentiation and the resulting chemical pathway leads to a significantly stronger natural enemy response than mechanical damage could.[11][12][13][14] The reliability of HIPVs in broadcasting the location of prey means that for many foraging enemies, induced plant volatiles are more attractive than even the odors emitted by the prey insect itself.[15]
Local and systemic signals
When herbivores trigger an inducible chemical defense pathway, the resulting HIPVs may be emitted from the site of feeding damage (local induction), or from undamaged tissues belonging to a damaged plant (systemic induction). For example, when an herbivore feeds on a single corn seedling leaf, the plant will emit volatiles from all its leaves, whether or not they too have been damaged. Locally induced defenses aid parasitoids in targeting their foraging behaviors to the exact location of the herbivore on the plant. Systemic defenses are less spatially specific and may serve to confuse the enemy once the source plant is located. A plant might employ both local and systemic responses simultaneously.[1]
Morphological mechanisms of enemy attraction
Domatia
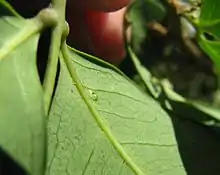
Natural enemies must survive long enough and respond quickly enough to plant volatiles in order to benefit the plant through predatory behavior. Certain plant structures, called domatia, can selectively reinforce mutualisms with natural enemies and increase the fitness benefit they receive from that mutualism by ensuring the survival and proximity of natural enemies. Domatia provide a kind of housing or refuge for predators from both abiotic stressors, such as desiccation, and biotic stress, such as predation from higher-order predators. Therefore, they ensure not only better survival, but eliminate the time required for natural enemies to locate and travel to the damaged plant. For this reason, natural enemies that make use of domatia are often said to serve as "bodyguards" for the plant in or on which they live. Domatia may be as well-developed as acacia tree thorns, or as simple and incidental as a depression or crevice in a leaf stem, but they are distinguishable from galls and other similar structures in that they are not induced by the insect but formed constitutively by the plant.[1]
Nutritional rewards
Plants are able to determine what types of herbivore species are present, and will react differently given the herbivore's traits. If certain defense mechanisms aren't effective, plants may turn to attracting natural enemies of herbivore populations. For example, wild tobacco plants use nicotine, a poison to defend against herbivores. However, when faced with nicotine-tolerant herbivores, they will attract the natural enemies of serious herbivores.[16] As long as the natural enemies have some potential to be omnivorous, plants can provide food resources to encourage their retention and increase the impact they have on herbivore populations. This potential, however, can hinge on a number of the insect's traits. For example, hemipteran predators can use their piercing-sucking mouthparts to make use of leaves, stems, and fruits, but spiders with chelicerae cannot.[17] Still, insects widely considered to be 100% carnivorous have recently been observed to diverge from expected feeding behavior.[18] Some plants simply tolerate a low level of herbivory by natural enemies for the service they provide in ridding the plant of more serious herbivores. Others, however, have structures thought to serve no purpose other than attracting and provisioning natural enemies. These structures derived from a long history of coevolution between the first and third trophic levels. A good example is the extrafloral nectaries which many myrmecophytes and other angiosperms sport on leaves, bracts, stems, and fruits. Nutritionally, extrafloral nectaries are similar to floral nectaries, but differ as they do not encourage the visiting insect to come into contact with pollen at any point. Their existence is therefore not the product of a pollinator-plant mutualism, but rather a tritrophic, defensive interaction.[17]
Human uses
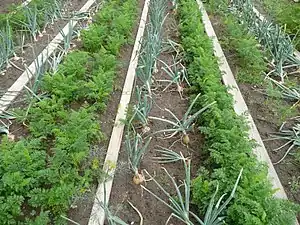
Systemic acquired resistance
Plants have shown they have the ability to build resistance to pathogens after initial infection. This ability is called systemic acquired resistance and comes to fruition after a plant has been infected by a pathogen. After surviving infection, the plant contains increased amounts of defense compounds such as chitinases. The increased presence of these defense compounds have shown to help prevent the plant from being affected again. Studies have shown that plants contain the ability to pass this resistance to their progeny.[20] An example of systemic acquired resistance was shown in Arabidopsis thaliana, in which the plants were exposed to fitness reducing levels of the bacterial disease PstDC3000. [20] After exposure, the subsequent 1st generation progeny of the diseased plant exhibited increase resistance to the disease PstDC3000. Exploitation of tritrophic interactions has the capacity to directly benefit agricultural systems. Significant biocontrol of crop pests can be exerted by the third trophic level, given an adequate population of natural enemies.[4][21] The widespread use of pesticides or Bt crops can undermine natural enemies’ success.[22][23][24] In some cases, entire populations of predators and parasitoids are decimated, necessitating even greater use of insecticide because the ecological service they provided in controlling herbivores has been lost.
Even when pesticides are not widely used, monocultures cannot support natural enemies in great enough numbers for them to have any impact on pest populations. A lack of diversity in the first trophic level is usually linked to low abundance in the third because alternative resources are missing from the system that are necessary for stable, large natural enemy populations. Enemy diets can be subsidized by increasing landscape diversity through companion planting, border crops, cover crops, intercropping, or allowing some weed growth.[19] When nectar or other sugar-rich resources are provided, the natural enemy population thrives.[25]
Morphological plant characteristics and natural enemy success
.jpg.webp)
Beyond domatia and nutritional rewards, there are numerous other plant characteristics that are involved in the successful colonization of natural enemies on plants. These can include the physical size, shape, density, maturity, colour, and texture of a given plant species. Specific plant features such as the hairiness or glossiness of vegetation can have mixed effects on different natural enemies. For example, trichomes decrease hunting efficiency in many natural enemies, as trichomes tend to slow or prevent movement due to the physical obstacles they present or due to the adhesive secretions they produce. However, this is not the case for all natural enemies. For example, E. formosa, a whitefly parasitoid is slowed by plant hairs which allows the parasitoid to detect and parasitize a higher number of juvenile whiteflies.[4]
Trichomes not only benefit the plant physically, but also chemically. Trichomes can develop in a variety of forms including, hairs or as pocket-like structures called glandular trichomes. These glandular trichomes store species-specific secondary metabolites such as terpenoids and phenolics in a pocket like structure between the cell wall and the cuticle of the plant. When glandular trichomes burst upon contact, they release these secondary metabolites and the strong odor and bitter taste of these substances cause insect herbivores to repel.[26] An example of trichomes used as a chemical defense against herbivores are found in the leaves of Urtica dioica. These leaves contain needle-like trichomes filled with a mix of histamine, oxalic acid, tartaric acid, formic acid, and serotonin which cause severe inflammation and irritation. These trichomes have a glassy looking tip which snaps off when it comes to contact with an herbivore and the needle-like trichome acts as a syringe, injecting the cocktail of histamines and acids into the skin.[26]
Studies have shown that trichome morphology and chemical composition has a role in herbivory response. The response of insect Helicoverpa sea on tomato plants Rutgers and Alisa Craig, as well as their respective mutants, was monitored in a study conducted by Donglan and his colleagues. They found a higher insect resistance on Alisa Craig, its mutant, and Rutger tomato plants treated with methyl jasmonate, a jasminate rich chemical which induces trichrome growth. There was low insect resistance on Rutger mutants which were also treated with methyl jasmonate. An adjacent study tested the trichome morphology of these four tomato plants and it was determined that Alisa Craig, its mutant and Rutger wild type tomato plants are rich in glandular trichomes while Rutger mutants are rich in non-glandular trichomes. Overall, this study suggests that glandular trichome density is an important factor of insect resistance.[27]
Trials involving Coccinelids have illustrated that many of these beetles have a distinct preference for the type of leaf surface they frequent. Presented with the opportunity to land on glossy or normal Brassica oleracea foliage, the beetles preferred inhabiting the glossy foliage as they were better able to grasp to these leaves. With this knowledge, many studies have been and are being conducted which evaluate the variation in effect on natural enemies when different plant genotypes are introduced.[4]
Volatile organic compounds
Two potential aspects that benefit plants when they release volatile organic compounds (VOCs) are the deterring of herbivores and the attraction of natural enemies; the latter has been a source of much research, investigating the relationship between natural enemies and VOCs as well as the resulting biological control.[28] There is the potential for synthesizing products which replicate the unique VOC composition released by different plants; these products could be applied to plants suffering from pests that are targeted by the attracted natural enemy.[28] This could cause natural enemies to enter crops that are occupied by pest populations that would otherwise likely remain undetected by the natural enemies.[29] The four elements that must be considered before manipulating VOCs are as follows: The VOCs must effectively aid the natural enemy in finding the prey; the pest must have natural enemies present; the fitness cost of potentially attracting additional herbivores must be exceeded by attracting enough natural enemies; and the natural enemies must not be negatively affected by direct plant defenses that may be present.[30]
Extrafloral nectaries
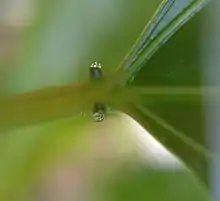
In a recent study comparing wild, cultivated, and nectarless cotton varieties in both the field and greenhouse, the findings demonstrated that the level of domestication of cotton plants correlates to the level of indirect defense investment, observed in the form of EFNs. It showed that wild varieties produced higher volumes of nectar and attracted a wider variety of natural enemies. This research points towards the idea that the process of breeding new cotton varieties has overlooked natural resistance traits in the pursuit of high yielding varieties that can be protected by pesticides. While this study did not find clear relationships between pest suppression and level of cotton domestication, it did highlight several studies which have shown this relationship. The studies illustrated a significant correlation between plants bearing EFNs and lower pest levels along with greater levels of natural enemies. One study also showed that the feeding of herbivores can directly induce nectar production.These collective findings illustrate the potential benefits that could be gained through incorporating the desirable genetics of wild varieties into cultivated varieties.[31]
Domatia
Certain tropical plants have been observed hosting colonies of ants in their hollow domatia and providing the ants with nutrition delivered from nectaries or food bodies. These ant colonies have become dependent on the host plants for their survival and, therefore, actively protect the plant; this protection can take the form of killing or warding off pests, weeds, and certain fungal pathogens. Chinese citrus farmers have capitalized on this mutualistic relationship for many years by incorporating artificial ant nests into their crops to suppress pests.[32]
Parasitoids
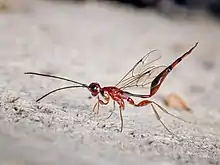
Parasitoids have successfully been incorporated into biological pest control programs for many years. It has been demonstrated that plants can directly influence the effect of parasitoids on herbivores by releasing chemical cues which alter host-seeking behavior and by providing food sources or domatia.[1] Certain parasitoids may be dependent on this plant relationship. Therefore, in production areas where parasitoid presence is desired, ensuring the crops being grown meet all of these requirements will likely correlate to higher parasitoid populations and potentially increased pest control.[29]
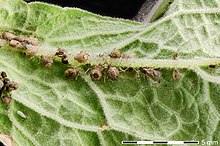
This has been illustrated through monitoring aphid populations in an experimental sugar beet crop. When only beets were grown, the parasitism level of the aphid population was insignificant. However, when collard crops were grown simultaneously and adjacent to the sugar beets, aphid parasitism levels increased. Collard crops released much higher concentrations of VOC’s compared to the sugar beets. As a result, it was found that the companion collard plants provided stronger chemical cues than the sugar beets. They attracted more aphid parasitoids which killed aphids in the collard plants and then migrated to the sugar beets that were in close proximity.[33]
In a related study, rice plants were observed to release ethylene and other compounds in response to brown plant hopper feeding, and this can attract a facultative parasitoid that parasitizes brown plant hopper eggs.[28]
In another study, it was shown that the presence of plant extraforal nectaries in cotton crops caused parasitoids to spend more time in them and caused the parasitization of more moth larva compared to cotton crops with no nectaries. Since the publication of this study, most farmers have transitioned to growing cotton varieties with nectaries.[34] In a separate trial involving cotton, it was revealed that naturalized cotton emitted seven times the VOC’s compared to cultivated cotton varieties when experiencing feeding damage.[35] This could be the case for other highly cultivated crop plants but must be measured, as there are cases of other crops that do not show the same trend.[29]
These findings reveal the specific variables a farmer can manipulate to influence parasitoid populations and illustrate the potential impact parasitoid habitat management can have on pest control.[29] In the case of cotton and other similar high VOC crop scenarios, there is interest in genetically engineering the chemical pathways of cultivated varieties to selectively produce the high VOC’s that were observed in the naturalized varieties in order to attract greater natural enemy populations. This presents numerous challenges but could produce promising pest control opportunities.[36]
Insect pathogens
.jpg.webp)
Insect pathogens, also called entomopathogens, are another group of organisms that are influenced by plants. The extent of the influence largely depends on the evolutionary history shared between the two as well as on the pathogens' method of infection and survival duration outside of a host. Several studies have shown that different insect host plants contain various compounds which cause different levels of insect mortality when certain entomopathogens are simultaneously injected. In some cases, significant increases in mortality are recorded, up to 50-fold in some studies. However, other research reveals that certain plants influence entomopathogens in negative ways, reducing their efficacy.[37]
It is primarily the leaf surface of the plant that influences the entomopathogen; plants can release various exudates, phytochemicals, and alleolochemicals through their leaves, some of which have the ability to inactivate certain entomopathogens.[37] In contrast, in other plant species, leaf characteristics can increase the efficacy of entomopathogens. For example, the mortality of pea aphids was higher in the group of aphids that were found on plants with fewer wax exudates compared to plants with more wax exudates. This reduced waxiness increased the transmission of Pandora neoaphidus conidia from the plant to the aphids.[38]
Feeding-induced volatiles that are emitted by different plants have been shown to increase the amount of spores released by certain entomopathogenic fungi, increasing the likelihood of infection of some herbivores but not others. Plants can also influence pathogen efficacy indirectly, and this typically occurs either by increasing the susceptibility of the herbivore hosts or by changing their behavior. This influence can often take the form of altered growth rates, herbivore physiology, or feeding habits. This research illustrates the differential impact that various host plant species can have on entomopathogenic interactions.[37]
In one study, it was shown that Brassicas can defend themselves by acting as a vector for entomopathogens. It was shown that virus-infected aphids feeding on the plants introduced a virus into the phloem. The virus was passively transported in the phloem and was carried throughout the plant. This caused the aphids that were feeding in separate locations from the infected aphids to become infected as well. This finding presents the possibility of injecting crops with compatible entomopathogenic viruses to defend against susceptible insect pests.[39]
Systemic acquired resistance
Plants have shown they have the ability to build resistance to pathogens after initial infection. This ability of plants to build a resistance to infection is called systemic acquired resistance and comes to fruition after a plant has been infected by a pathogen. After surviving infection, the plant contains increased amounts of defense compounds such as chitinases. The increased presence of these defense compounds have shown to help prevent the plant from being affected again. Studies have also shown that plants contain the ability to pass this resistance to their progeny.[40] An example of systemic acquired resistance was shown in Arabidopsis thaliana, in which the plants were exposed to fitness reducing levels of the bacterial disease PstDC3000. [40] After exposure, the subsequent first-generation progeny of the diseased plant exhibited increase resistance when exposed to the disease PstDC3000. [40] In this study, while increased resistance for the disease PstDC3000 was found, it is important to note that the progeny had an increased susceptibility to a necrotrophic fungus called Alternaria brassicicola. [40] The progeny also had a decreased response to the hormone jasmonic acid. [40]
Below-ground tritrophic interactions
Less studied than above-ground interactions, but proving to be increasingly important, are the below-ground interactions that influence plant defense.[41] There is a complex network of signal-transduction pathways involved in plant responses when they are receiving various stimuli, and it has been shown that soil microbes have a significant influence on a number of these responses. Certain soil microbes can aid plant growth, producing increased tolerance to various environmental stressors, and can protect their host plants from many different pathogens by inducing systemic resistance.[42] It is understood that organisms in above- and below-ground environments can interact indirectly through plants. Many studies have shown both the positive and negative effects that one organism in one environment can have on other organisms in the same or opposite environment, with the plant acting as the intermediary between the two.[41]
.jpg.webp)
A meta analysis documenting the effect of mycorhizae demonstrated this. The colonization of plant roots with mycorhizae typically results in a mutualistic relationship between the plant and the fungus, inducing a number of changes in the plant. The analysis reviewed the mixed impact that colonization can have on herbivores; it highlighted that insects with different feeding methods were affected very differently, some positively and others negatively. The type of mycorhizae species involved also had a notable impact on the performance of the insects. One common species, Glomus intradices, had a negative effect on the feeding success of chewing herbivores, whereas other species studied had positive effects.[43]
One study showed that the roots of some maize plants produce a defense chemical when roots are damaged by leaf beetle larvae; this chemical was very attractive to the entomopathogenic nematode species Heterorhabditis megidis. It was found that only certain maize varieties produce this chemical; in the field, plants which released the chemical caused up to a five-fold increase in leaf beetle larvae parasitization over those that didn't produce the chemical. Incorporating these varieties or their genes into commercial maize production could significantly increase the efficacy of nematode treatments.[44]
Expanding on this study, in a further experiment, additional nematodes, herbivores and plant species were compared in order to record resulting interactions. The underlying findings suggested that the plant-emitted chemicals acted as the primary source of attractant to the nematodes. It was suggested that the herbivores have evolved to be relatively undetectable to the nematodes, whereas the plants have evolved to release highly attractive chemical signals. It is clear that a high degree of specificity is involved; species that make up these tritrophic interactions have evolved closely with one another over a long period of time and as a result have very unique relationships.[45]
An additional experiment showed that the bacterium E. aerogenes produces the volatile 2,3-butanediol which had impacts on interactions between plants, pathogens, and insects.[46] When maize plants were grown in a soil culture containing the volatile bacterium or inoculated with the bacterium in its plant mass, researchers found that the maize were more resistant to the pathogen Setosphaeria turcica as there was a significant decrease in neurotic development and hyphae length when compared to bacteria absent maize.[46] Furthermore, researchers found that the bacterium did not deter insect herbivory, rather it increased weight gain and leaf consumption in a caterpillar species known as Spodoptera littoralis [46] . Lastly, when observing the attraction of a natural predator known as Cotesia marginiventris researchers found that these wasps were attracted more readily to maize plants that were grown in soil cultures containing either volatile producing bacterium or pure 2,3-butanediol.[46] These results confirm the idea that soil microorganism does play a role in influencing tritrophic interactions between plants and insects.
Considerations in utilizing tritrophic interactions in biological control
Sustainable crop production is becoming increasingly important, if humans are to support a growing population and avoid a collapse of production systems.[47] While the understanding and incorporation of tritrophic interactions in pest control offers a promising control option, it is important to note that the sustainable biological control of pests requires a dynamic approach that involves diversity in all of the species present, richness in natural enemies, and limited adverse activity (i.e., minimal pesticide use). This approach is especially important in conservation biological control efforts.[48] It is also important to note that there are typically more than three trophic levels at play in a given production setting, so it has been suggested that the tritrophic interaction research model is somewhat simplistic and should, in some cases, incorporate the influence that higher trophic levels can have on biological control.[29] Furthermore, ecological complexity and interactions between species of the same trophic level are topics that still require much research. Up to this point, the research has had a relatively narrow focus, which may be suitable for controlled environments such as greenhouses but which is lacking in its documentation of multi-generational plant interactions with dynamic communities of organisms.[49]
References
- Price, Peter W. (2011). Insect Ecology : Behavior, Populations and Communities (4th ed.). Cambridge: Cambridge University Press. ISBN 978-0-521-83488-9.
- Heil, Martin (2008). "Indirect defence via tritrophic interactions". New Phytologist. 178 (1): 41–61. doi:10.1111/j.1469-8137.2007.02330.x. PMID 18086230.
- Karban, Richard (2011). "The ecology and evolution of induced resistance against herbivores". Functional Ecology. 25 (2): 339–347. doi:10.1111/j.1365-2435.2010.01789.x.
- Bottrell, Dale G.; Barbosa, Pedro; Gould, Fred (1998). "Manipulating Natural Enemies By Plant Variety Selection and Modification: A Realistic Strategy?". Annual Review of Entomology. 43 (1): 347–367. doi:10.1146/annurev.ento.43.1.347. PMID 15012394.
- Fraenkel, G. S. (29 May 1959). "The Raison d'Etre of Secondary Plant Substances: These odd chemicals arose as a means of protecting plants from insects and now guide insects to food". Science. 129 (3361): 1466–1470. Bibcode:1959Sci...129.1466F. doi:10.1126/science.129.3361.1466. PMID 13658975.
- Whittaker, R. H.; Feeny, P. P. (26 February 1971). "Allelochemics: Chemical Interactions between Species". Science. 171 (3973): 757–770. Bibcode:1971Sci...171..757W. doi:10.1126/science.171.3973.757. PMID 5541160.
- Taiz, Lincoln (2018). Fundamentals of Plant Physiology. 198 Madison Avenue, New York, New York 10016 United States: Oxford University Press. pp. 520–521.CS1 maint: location (link)
- Rasmann, Sergio; Köllner, Tobias G.; Degenhardt, Jörg; Hiltpold, Ivan; Toepfer, Stefan; Kuhlmann, Ulrich; Gershenzon, Jonathan; Turlings, Ted C. J. (7 April 2005). "Recruitment of entomopathogenic nematodes by insect-damaged maize roots" (PDF). Nature. 434 (7034): 732–737. Bibcode:2005Natur.434..732R. doi:10.1038/nature03451. PMID 15815622. S2CID 4104266.
- Frost, Christopher J.; Appel, Heidi M.; Carlson, John E.; De Moraes, Consuelo M.; Mescher, Mark C.; Schultz, Jack C. (2007). "Within-plant signalling via volatiles overcomes vascular constraints on systemic signalling and primes responses against herbivores". Ecology Letters. 10 (6): 490–498. doi:10.1111/j.1461-0248.2007.01043.x. PMID 17498148.
- Kessler, D.; Gase, K.; Baldwin, I. T. (29 August 2008). "Field Experiments with Transformed Plants Reveal the Sense of Floral Scents". Science. 321 (5893): 1200–1202. Bibcode:2008Sci...321.1200K. doi:10.1126/science.1160072. PMID 18755975. S2CID 206513974.
- Turlings, T. C. J.; Tumlinson, J. H.; Lewis, W. J. (30 November 1990). "Exploitation of Herbivore-Induced Plant Odors by Host-Seeking Parasitic Wasps" (PDF). Science. 250 (4985): 1251–1253. Bibcode:1990Sci...250.1251T. doi:10.1126/science.250.4985.1251. PMID 17829213. S2CID 13430669.
- Mattiacci, Letizia; Dicke, Marcel; Posthumus, Maarten A. (1994). "Induction of parasitoid attracting synomone in brussels sprouts plants by feeding ofPieris brassicae larvae: Role of mechanical damage and herbivore elicitor". Journal of Chemical Ecology. 20 (9): 2229–2247. doi:10.1007/BF02033199. PMID 24242803. S2CID 6121052.
- Halitschke, R.; Keßler, A.; Kahl, J.; Lorenz, A.; Baldwin, I. T. (23 August 2000). "Ecophysiological comparison of direct and indirect defenses in Nicotiana attenuata". Oecologia. 124 (3): 408–417. Bibcode:2000Oecol.124..408H. doi:10.1007/s004420000389. PMID 28308780. S2CID 5034158.
- Röse, Ursula S. R.; Tumlinson, James H. (22 April 2005). "Systemic induction of volatile release in cotton: How specific is the signal to herbivory?". Planta. 222 (2): 327–335. doi:10.1007/s00425-005-1528-2. PMID 15856281. S2CID 13438362.
- Steinberg, Shimon; Dicke, Marcel; Vet, Louise E. M. (1993). "Relative importance of infochemicals from first and second trophic level in long-range host location by the larval parasitoidCotesia glomerata". Journal of Chemical Ecology. 19 (1): 47–59. doi:10.1007/BF00987470. PMID 24248510. S2CID 797047.
- Taiz, Lincoln (2018). Fundamentals of Plant Physiology. New York, NY: Oxford University Press USA. p. 511.
- Price, Peter W. (2011). Insect Ecology : Behavior, Populations and Communities (4th ed.). Cambridge: Cambridge University Press. ISBN 978-0-521-83488-9.
- Rubinoff, D. (22 July 2005). "Web-Spinning Caterpillar Stalks Snails". Science. 309 (5734): 575. doi:10.1126/science.1110397. PMID 16040699. S2CID 42604851.
- Landis, Douglas A.; Wratten, Stephen D.; Gurr, Geoff M. (2000). "Habitat Management to Conserve Natural Enemies of Arthropod Pests in Agriculture". Annual Review of Entomology. 45 (1): 175–201. doi:10.1146/annurev.ento.45.1.175. PMID 10761575.
- Luna, Estrella; Bruce, Toby J. A.; Roberts, Michael R.; Flors, Victor; Ton, Jurriaan (2012-02-01). "Next-Generation Systemic Acquired Resistance". Plant Physiology. 158 (2): 844–853. doi:10.1104/pp.111.187468. ISSN 0032-0889. PMC 3271772. PMID 22147520.
- Cortesero, A.M.; Stapel, J.O.; Lewis, W.J. (2000). "Understanding and Manipulating Plant Attributes to Enhance Biological Control". Biological Control. 17 (1): 35–49. CiteSeerX 10.1.1.337.1094. doi:10.1006/bcon.1999.0777.
- Obrycki, John J. (2001). "Transgenic Insecticidal Corn: Beyond Insecticidal Toxicity to Ecological Complexity Analysis of transgenic insecticidal corn developed for lepidopteran pests reveals that the potential benefits of crop genetic engineering for insect pest management may not outweigh the potential ecological and economic risks". BioScience. 51 (5): 353–361. doi:10.1641/0006-3568(2001)051[0353:TICBIT]2.0.CO;2.
- Groot, Astrid T.; Dicke, Marcel (2002). "Insect-resistant transgenic plants in a multi-trophic context". The Plant Journal. 31 (4): 387–406. doi:10.1046/j.1365-313X.2002.01366.x. PMID 12182699.
- Poppy, Guy M.; Sutherland, Jamie P. (2004). "Can biological control benefit from genetically-modified crops? Tritrophic interactions on insect-resistant transgenic plants". Physiological Entomology. 29 (3): 257–268. doi:10.1111/j.0307-6962.2004.00382.x. S2CID 59330885.
- Wäckers, edited by F.L.; Rijn, P.C.J. van; Bruin, J. (2005). Plant-provided food for carnivorous insects : protective mutualism and its applications (1. paperback ed.). New York: Cambridge University Press. ISBN 978-0521819411.CS1 maint: extra text: authors list (link)
- Taiz, Lincoln (2018). Fundamentals of Plant Physiology. New York, NY: Oxford University Press USA. pp. 511–512.
- Tian, Donglan; Tooker, John; Peiffer, Michelle; Chung, Seung Ho; Felton, Gary W. (October 2012). "Role of trichomes in defense against herbivores: comparison of herbivore response to woolly and hairless trichome mutants in tomato (Solanum lycopersicum)". Planta. 236 (4): 1053–1066. doi:10.1007/s00425-012-1651-9. ISSN 1432-2048. PMID 22552638. S2CID 14093399.
- Nurindah, N., Wonorahardjo, S., Sunarto, D. A., Sujak, S. (2017). "Chemical Cues In Tritrophic Interactions On Biocontrol Of Insect Pest". The Journal of Pure and Applied Chemistry Research. 6 (1): 49–56. doi:10.21776/ub.jpacr.2017.006.01.282.CS1 maint: multiple names: authors list (link)
- Poppy, G. M. (1997). "Tritrophic interactions: Improving ecological understanding and biological control?". Endeavour. 21 (2): 61–65. doi:10.1016/S0160-9327(97)01042-9.
- Kessler, A., Baldwin, I. T. (2002). "Plant-Mediated Tritrophic Interactions and Biological Pest Control". AgBiotechNet. 4 – via Research Gate.CS1 maint: multiple names: authors list (link)
- Llandres, A. L., Verdeny-Vilalta, O., Jean, J., Goebel, F., Seydi, O., Brevault, T. (2019). "Cotton Extrafloral Nectaries as Indirect Defence Against Insect Pests". Basic and Applied Ecology. 37: 24–34. doi:10.1016/j.baae.2019.05.001 – via Elsevier Science Direct.CS1 maint: multiple names: authors list (link)
- Heil, M. (2008). "Indirect Defence via Tritrophic Interactions". The New Phytologist. 178 (1): 41–61. doi:10.1111/j.1469-8137.2007.02330.x. PMID 18086230 – via JSTOR.
- Read, D. P., Feeny, P. P., Root, R. B. (1970). "Habitat Selection By The Aphid Parasite Diaeretiella Rapae (Hymenoptera: Braconidae) And Hyperparasite Charips Brassicae (Hymenoptera: Cynipidae)". The Canadian Entomologist. 102 (12): 1567–1578. doi:10.4039/Ent1021567-12.CS1 maint: multiple names: authors list (link)
- Stapel, J. O., Cortesero, A. M., DeMoraes, C. R., Tumlinson, J. H. and Lewis, W. J. (1996). "Extrafloral Nectar, Honeydew, and Sucrose Effects on Searching Behavior and Efficiency of Microplitis croceipes (Hymenoptera: Braconidae) in Cotton". Environmental Entomology. 26 (3): 617–623. doi:10.1093/ee/26.3.617.CS1 maint: multiple names: authors list (link)
- Loughrin, J. H., Manukian, A., Heath, R. R., Tumlinson, J. H. (1995). "Volatiles Emitted by Different Cotton Varieties Damaged by Feeding Beet Armyworm Larvae". Chemical Ecology. 21 (8): 1217–1227. doi:10.1007/BF02228321. PMID 24234527. S2CID 35273594.CS1 maint: multiple names: authors list (link)
- Poppy, Guy M.; Sutherland, Jamie P. (2004). "Can biological control benefit from genetically‐modified crops? Tritrophic interactions on insect‐resistant transgenic plants". Physiological Entomology. 29 (3): 257–268. doi:10.1111/j.0307-6962.2004.00382.x. S2CID 59330885.CS1 maint: multiple names: authors list (link)
- Cory, J. S., Hoover, K. (2006). "Plant-Mediated Effects in Insect-Pathogen Interactions". Trends in Ecology & Evolution. 21 (5): 278–286. doi:10.1016/j.tree.2006.02.005. PMID 16697914.CS1 maint: multiple names: authors list (link)
- Duetting, P. S., Eigenbrode, S. D. (2003). "Plant Waxy Bloom on Peas Affects Infection of Pea Aphids by Pandora neoaphidus". Invertebrate Pathology. 84 (3): 149–158. doi:10.1016/j.jip.2003.10.001. PMID 14726238.CS1 maint: multiple names: authors list (link)
- Van Munster, M., Janssen, A., Clerivet, A., Van Den Heuvel, J. (2005). "Can Plants Use an Entomopathogenic Virus as a Defense Against Herbivores?". Plant Animal Interactions. 143 (3): 396–401. Bibcode:2005Oecol.143..396V. doi:10.1007/s00442-004-1818-6. PMID 15723235. S2CID 29076492.CS1 maint: multiple names: authors list (link)
- Luna, Estrella; Bruce, Toby J. A.; Roberts, Michael R.; Flors, Victor; Ton, Jurriaan (2012-02-01). "Next-Generation Systemic Acquired Resistance". Plant Physiology. 158 (2): 844–853. doi:10.1104/pp.111.187468. ISSN 0032-0889. PMC 3271772. PMID 22147520.
- Van Geem, M., Gols, R., Van Dam, N. M., Van Der Putten, W. H., Fortuna, T., Harvey, J. A. (2013). "The Importance of Aboveground–Belowground Interactions on the Evolution and Maintenance of Variation in Plant Defense Traits". Frontiers in Plant Science. 28: 431. doi:10.3389/fpls.2013.00431. PMC 3842511. PMID 24348484.CS1 maint: multiple names: authors list (link)
- Pineda, A., Dicke, M., Pieterse, C. M. J., Pozo, M. J. (2013). "Beneficial microbes in a changing environment: are they always helping plants to deal with insects?". Functional Ecology. 27 (3): 574–586. doi:10.1111/1365-2435.12050. hdl:1874/276314.CS1 maint: multiple names: authors list (link)
- Koricheva, J., Gange, A. C., Jones, T. (2009). "Effects of Mycorrhizal Fungi on Insect Herbivores: A Meta-Analysis". Ecology. 90 (8): 2088–2097. doi:10.1890/08-1555.1. PMID 19739371 – via NCBI PubMed.CS1 maint: multiple names: authors list (link)
- Rasmann, S., Kollner, T. G., Degenhardt, J., Hiltpold, I., Toepfer, S., Kuhlmann, U., Gershenzon, J., Turlings, T. C. J. (2005). "Recruitment of Entomopathogenic Nematodes by Insect-Damaged Maize Roots". Nature. 434 (7034): 732–737. Bibcode:2005Natur.434..732R. doi:10.1038/nature03451. PMID 15815622. S2CID 4104266.CS1 maint: multiple names: authors list (link)
- Rasmann, S., Turlings, T. C. J. (2008). "First Insights Into Specificity of Belowground Tritrophic Interactions" (PDF). OIKOS. 117 (3): 362–369. doi:10.1111/j.2007.0030-1299.16204.x.CS1 maint: multiple names: authors list (link)
- Alessandro, Marco (2014). "Volatiles produced by soil-borne endophytic bacteria increase plant pathogen resistance and affect tritrophic interactions". Plant, Cell and Environment. 37 (4): 813–826. doi:10.1111/pce.12220. PMC 4194311. PMID 24127750.
- Berg, G. (2009). "Plant–Microbe Interactions Promoting Plant Growth and Health: Perspectives for Controlled Use of Microorganisms in Agriculture". Applied Microbiology and Biotechnology. 84 (1): 11–18. doi:10.1007/s00253-009-2092-7. PMID 19568745. S2CID 3330216.
- Gardarin, A., Plantegenest, M., Bischoff. A., Valantin-Morison, M. (2018). "Understanding plant–arthropod interactions in multitrophic communities to improve conservation biological control: useful traits and metrics" (PDF). Journal of Pest Science. 91 (3): 943–955. doi:10.1007/s10340-018-0958-0. S2CID 43962364.CS1 maint: multiple names: authors list (link)
- Van Veen, F. (2015). "Plant-modified Trophic Interactions". Current Opinion in Insect Science. 8: 29–33. doi:10.1016/j.cois.2015.02.009. hdl:10871/17566. PMID 32846667 – via Elsevier Science Direct.