Crystal engineering
Crystal engineering is the design and synthesis of molecular solid state structures with desired properties, based on an understanding and use of intermolecular interactions. The two main strategies currently in use for crystal engineering are based on hydrogen bonding and coordination bonding. These may be understood with key concepts such as the supramolecular synthon and the secondary building unit.

History of term
The term 'crystal engineering' was first used in 1955 by R. Pepinsky [1] but the starting point is often credited to Gerhard Schmidt[2] in connection with photodimerization reactions in crystalline cinnamic acids. Since this initial use, the meaning of the term has broadened considerably to include many aspects of solid state supramolecular chemistry. A useful modern definition is that provided by Gautam Desiraju, who in 1988 defined crystal engineering as "the understanding of intermolecular interactions in the context of crystal packing and the utilization of such understanding in the design of new solids with desired physical and chemical properties."[3] Since many of the bulk properties of molecular materials are dictated by the manner in which the molecules are ordered in the solid state, it is clear that an ability to control this ordering would afford control over these properties.
Non-covalent control of structure
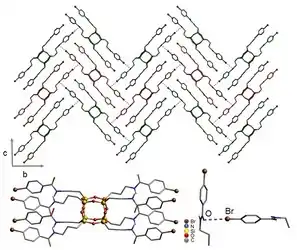
Crystal engineering relies on noncovalent bonding to achieve the organization of molecules and ions in the solid state. Much of the initial work on purely organic systems focused on the use of hydrogen bonds, though with the recent extension to inorganic systems, the coordination bond has also emerged as a powerful tool. In addition to this, especially through studies during the last decade, the use of halogen bonds has proved beneficial in providing additional control in crystal design.[5] Other intermolecular forces such as π...π and Au...Au interactions have all been exploited in crystal engineering studies, and ionic interactions can also be important. However, the two most common strategies in crystal engineering still employ only hydrogen bonds and coordination bonds.
Molecular self-assembly is at the heart of crystal engineering, and it typically involves an interaction between complementary hydrogen bonding faces or a metal and a ligand. By analogy with the retrosynthetic approach to organic synthesis, Desiraju coined the term "supramolecular synthon"[6] to describe building blocks that are common to many structures and hence can be used to order specific groups in the solid state.[7] The carboxylic acid dimer represents a simple supramolecular synthon, though in practice this is only observed in approximately 30% of crystal structures in which it is theoretically possible. The Cambridge Structural Database (CSD) provides an excellent tool for assessing the efficiency of particular synthons. The supramolecular synthon approach has been successfully applied in the synthesis of one dimensional tapes, two dimensional sheets and three dimensional structures. The CSD today contains atomic positional parameters for nearly 800 000 crystal structures, and this forms the basis for heuristic or synthon based or "experimental" crystal engineering.
Design of multi-component crystals
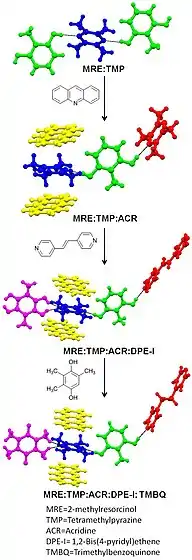
A major development in the field of crystal engineering in the last decade is related to the development of design strategies for bi-component and higher multi-component crystals (also known as cocrystals). The design of cocrystals is a difficult task as it involves recognition between different molecules which might be completely different in shape and size. Therefore, the more the number of components in a crystal, the more challenging it is to synthesize. Initially, the synthesis of cocrystals was centered on the design of binary ones. This is most often achieved with strong heteromolecular interactions. Ternary ones were designed mainly by interaction insulation, interaction hierarchy[8] or by shape-size mimicry.[9] However, it has been shown recently that it is possible to synthesize up to five-component crystals by choosing a suitable retrosynthetic strategy.[10] The main relevance of multi-component crystals, apart from the synthetic challenge, arises from the advantage of tuning a particular property by changing the components. The main development in this front is focused upon designing pharmaceutical cocrystals.[11] Pharmaceutical cocrystals are generally composed of one API (Active Pharmaceutical Ingredient) with other molecular substances that are considered safe according to the guidelines provided by WHO (World Health Organization). It has been shown that various properties (such as solubility, bioavailability, permeability) of an API can be modulated through the formation of pharmaceutical cocrystals.
In two dimensions
The study and formation of 2D architectures (i.e., molecularly thick architectures) has rapidly emerged as a branch of engineering with molecules.[12] The formation (often referred as molecular self-assembly depending on its deposition process) of such architectures lies in the use of solid interfaces to create adsorbed monolayers. Such monolayers may feature spatial crystallinity in an investigated time window, and thus the terminology of 2D crystal engineering is well suited.[13][14] However the dynamic and wide range of monolayer morphologies ranging from amorphous to network structures have made of the term (2D) supramolecular engineering a more accurate term. Specifically, supramolecular engineering refers to "(The) design (of) molecular units in such way that a predictable structure is obtained"[15] or as "the design, synthesis and self-assembly of well defined molecular modules into tailor-made supramolecular architectures".[16]
The field of 2D crystal engineering has advanced over the years especially through the advent of scanning probe microscopic techniques which enable one to visualize networks with sub-molecular precision. Understanding the mechanism of these two dimensional assemblies may provide insights to the bottom up fabrication processes at the interfaces. The many aspects of developments in this field include the understanding of interactions, studies on polymorphism, design of nanoporous networks. Engineering the size and symmetry of the cavities and performing host guest chemistry inside the pores under nano confinement remains an attractive interest of this field. More recently, multicomponent networks are also studied that are formed by the application of crystal engineering principles. Although, there is a very high influence of the underlying substrate on the formation of the two dimensional assemblies, at least in a few cases, a relation was found between 2D assemblies and the bulk crystal structures.
Polymorphism
Polymorphism is the phenomenon wherein the same chemical compound exists in different crystal forms. In the initial days of crystal engineering, polymorphism was not properly understood and incompletely studied. Today, it is one of the most exciting branches of the subject partly because polymorphic forms of drugs may be entitled to independent patent protection if they show new and improved properties over the known crystal forms. With the growing importance of generic drugs, the importance of crystal engineering to the pharmaceutical industry is expected to grow exponentially.[17]
Polymorphism arises due to the competition between kinetic and thermodynamic factors during crystallization. While long-range strong intermolecular interactions dictate the formation of kinetic crystals, the close packing of molecules generally drives the thermodynamic outcome. Understanding this dichotomy between the kinetics and thermodynamics constitutes the focus of research related to the polymorphism.

In organic molecules, three types of polymorphism are mainly observed. Packing polymorphism arises when molecules pack in different ways to give different structures. Conformational polymorphism, on the other hand is mostly seen in flexible molecules where molecules have multiple conformational possibilities within a small energy window. As a result, multiple crystal structures can be obtained with the same molecule but in different conformations. The rarest form of polymorphism arises from the differences in the primary synthon and this type of polymorphism is called as synthon polymorphism. With the growth in research in the cocrystals in recent times, it is observed that cocrystals are also prone to polymorphism.
Crystal structure prediction
Crystal structure prediction (CSP) is a computational approach to generate energetically feasible crystal structures (with corresponding space group and positional parameters) from a given molecular structure. The CSP exercise is considered most challenging as "experimental" crystal structures are very often kinetic structures and therefore are very difficult to predict. In this regard, many protocols have been proposed and are tested through several blind tests organized by CCDC since 2002. A major advance in the CSP happened in 2007 while a hybrid method based on tailor made force fields and density functional theory (DFT) was introduced. In the first step, this method employs tailor made force fields to decide upon the ranking of the structures followed by a dispersion corrected DFT method to calculate the lattice energies precisely.[18]
Apart from the ability of predicting crystal structures, CSP also gives computed energy landscapes of crystal structures where many structures lie within a narrow energy window.[19] This kind of computed landscapes lend insights into the study on polymorphism, design of new structures and also help to design crystallization experiments.
Property design

The design of crystal structures with desired properties is the ultimate goal of crystal engineering. While major advances towards this have been made in coordination polymers, the application of successful strategies to design properties in purely organic solids is still limited. Most of the initial studies related to the crystal engineering of organic molecular solids were concentrated upon understanding the intermolecular interaction and identification of supramolecular synthons. In recent years, considerable attention has been given to the design the organic solids with specific properties. The early attempts to apply crystal engineering principles to design the functional solids were focused upon the synthesis of non-linear optical materials, especially those with second harmonic generation (SHG) properties. By designing supramolecular synthons, supramolecular gels,[20][21] and corrdination polymer based gel can also be designed.[22]
Another major development in this area is related to the control of photodimerization reactions through templates by application of crystal engineering strategies. The importance of topochemical control in solid-state reactions was first realized by Schmidt. However, with the advent of cocrystals, multiple strategies have been discovered over the years to get photodimerization products from the molecules which are otherwise photo inactive with precise stereochemistry.[23] A more recent development in property design is linked with the mechanical properties in relation with the crystal structures.[24] Various mechanical properties such as bending, shearing or brittleness have been explained on the basisy of crystal packing. It has also been shown that solids of desired mechanical properties can be designed through the precise control of packing features. More recently, nanoindentation techniques are used in quantification of some of these properties in terms of hardness and elasticity.[25]
Apart from these properties, significant efforts have been made to control the photophysical properties as well. Despite all the developments made so far, this area of property design, especially in the purely organic solids, is still at an evolving stage and needs more directed approaches to understand the connectivity between the crystal structures and their respective properties.
A holistic view
Crystallization mechanism forms the core of all the problems in crystal engineering. A proper understanding of crystallization mechanisms will enable us to sort among the various possibilities generated by say CSP. Moreover, it will help a crystal engineer to design a solid with particular function.[26] It is therefore of immense importance to understand the progression from solution to the nucleus to the crystal structures, in other terms, how the entropy dominated situation in solution converts to an enthalpy driven one in the crystals through the nucleation step. As the nucleus is difficult to identify, the approaches made towards this end can be generally divided into two categories. The first type centers on the studies in solution through various spectroscopic techniques to understand the structure of the assembly in the solution and these studies give an idea about the initial stages of crystallization.[27] The second type of approach take into account all the relevant crystal structures of a given compound and this constitutes a structural landscape.[28] Each structure in a structural landscape is considered as an individual data point and while taken together it provides a holistic viewpoint towards the crystallization mechanism. A special situation happens when multiple components are observed in an asymmetric unit (Z′>1). These structures result from the interrupted crystallization and therefore shed lights on the intermediate stages of crystallization.
Specialized journals
Crystal engineering is a rapidly expanding discipline as revealed by the recent appearance of several international scientific journals in which the topic plays a major role. These include CrystEngComm from the Royal Society of Chemistry and Crystal Growth & Design from the American Chemical Society. The new open access journals IUCrJ from the International Union of Crystallography and Crystals from MDPI have crystal engineering as one of their main sections, reflecting the importance of this subject in modern structural chemistry.
See also
External links
![]() |
Wikimedia Commons has media related to Crystal engineering. |
References
- R. Pepinsky, Crystal Engineering - New Concept in Crystallography, Physical Review , 1955, 100, 971.
- G. M. J. Schmidt, Photodimerization in the solid state, Pure Appl. Chem., 1971, 27, 647.
- G. R. Desiraju, Crystal Engineering: The design of Organic Solids, Elsevier, 1989, Amsterdam
- Janeta, Mateusz; Szafert, Sławomir (2017-10-01). "Synthesis, characterization and thermal properties of T8 type amido-POSS with p-halophenyl end-group". Journal of Organometallic Chemistry. 847: 173–183. doi:10.1016/j.jorganchem.2017.05.044. ISSN 0022-328X.
- P. Metrangolo, H. Neukirch, T. Pilati and G. Resnati, Halogen Bonding Based Recognition Processes: A World Parallel to Hydrogen Bonding, Acc. Chem. Res. 2005, 38, 386-395.
- G. R. Desiraju, Supramolecular Synthons in Crystal Engineering—A New Organic Synthesis, Angew. Chem. Int. Ed. 1995, 34, 2311-2327.
- A. Nangia and G. R. Desiraju, Supramolecular Structures - Reason and Imagination, Acta Crystallogr. 1998, A54, 934-944.
- C. B. Aakeröy, A. M. Beatty and B. A. Helfrich, "Total Synthesis" Supramolecular Style: Design and Hydrogen-Bond-Directed Assembly of Ternary Supermolecules, Angew. Chem. Int. Ed. 2001, 40, 3240-3242.
- S. Tothadi, A. Mukherjee and G. R. Desiraju, Shape and size mimicry in the design of ternary molecular solids: towards a robust strategy for crystal engineering, Chem. Commun. 2011, 47, 12080-12082.
- N. A. Mir, R. Dubey and G. R. Desiraju, Four- and five-component molecular solids: crystal engineering strategies based on structural inequivalence, IUCrJ 2016, 3, 96-101.
- O. Almarsson and M. J. Zaworotko, Crystal engineering of the composition of pharmaceutical phases. Do pharmaceutical co-crystals represent a new path to improved medicines?, Chem. Commun. 2004, 1889-1896
- J. V. Barth, G. Constantini, K. Kern, Engineering atomic and molecular nanostructures at surfaces, Nature, 2005, 437, 671–679.
- C.A. Palma, M. Bonini, T. Breiner, P. Samori, Supramolecular Crystal Engineering at the Solid– Liquid Interface from First Principles: Toward Unraveling the Thermodynamics of 2D Self- Assembly, Adv. Mat., 2009, 21, 1383–1386
- J. A. A. W. Elemans, S.B. Lei S. De Feyter, Molecular and Supramolecular Networks on Surfaces: From Two Dimensional Crystal Engineering to Reactivity, Angew. Chem. Int. Ed., 2009, 48, 7298–7332
- J. Simon, P. Bassoul, Design of molecular materials: supramolecular engineering, 2000 WileyVCH
- A. Ciesielski, C.A. Palma, M. Bonini, P. Samori, Towards Supramolecular Engineering of Functional Nanomaterials: PreProgramming MultiComponent 2D SelfAssembly at Solid Liquid Interfaces, Adv. Mat., 2010, 22, 3506–3520.
- D. Braga, F. Grepioni, L. Maini and M. Polito in Crystal Polymorphism and Multiple Crystal Forms, Vol. (Ed. W. M. Hosseini), Springer Berlin Heidelberg, Berlin, Heidelberg, 2009, pp. 87-95.
- M. A. Neumann, F. J. J. Leusen and J. Kendrick, A Major Advance in Crystal Structure Prediction, Angew. Chem. Int. Ed. 2008, 47, 2427-2430.
- S. L. Price, Computed Crystal Energy Landscapes for Understanding and Predicting Organic Crystal Structures and Polymorphism, Acc. Chem. Res. 2009, 42, 117–126.
- P. Dastidar, Supramolecular gelling agents: can they be designed? Chem. Soc. Rev. 2008, 37, 2699
- P. Sahoo, D. K. Kumar, S. R. Raghavan, P. Dastidar. Supramolecular Synthons in Designing Low Molecular Mass Gelling Agents: L-Amino Acid Methyl Ester Cinnamate Salts and their Anti-Solvent-Induced Instant Gelation Chem. Asian J. 2011, 6, 1038–1047
- P. Sahoo, N. N. Adarsh, P. Dastidar, Is a Crystal Engineering Approach Useful in Designing Metallogels? A Case Study. Cryst. Growth Des. 2010, 10, 11, 4976–4986
- L. R. MacGillivray, J. L. Reid and J. A. Ripmeester, Supramolecular Control of Reactivity in the Solid State Using Linear Molecular Templates, J. Am. Chem. Soc. 2000, 122, 7817-7818.
- C. M. Reddy, R. C. Gundakaram, S. Basavoju, M. T. Kirchner, K. A. Padmanabhan and G. R. Desiraju, Structural basis for bending of organic crystals, Chem. Commun. 2005, 3945-3947.
- S. Varughese, M. S. R. N. Kiran, U. Ramamurty and G. R. Desiraju, Nanoindentation in Crystal Engineering: Quantifying Mechanical Properties of Molecular Crystals, Angew. Chem. Int. Ed. 2013, 52, 2701-2712.
- G. R. Desiraju, Crystal Engineering: A Holistic View, Angew. Chem. Int. Ed. 2007, 46, 8342–8356.
- S. Parveen, R. J. Davey, G. Dent and R. G. Pritchard, Linking solution chemistry to crystal nucleation: the case of tetrolic acid, Chem. Commun. 2005, 1531–1533.
- G. R. Desiraju, Crystal Engineering: From Molecule to Crystal, J. Am. Chem. Soc. 2013, 135, 9952–9967.